Meridional Modes and their indices
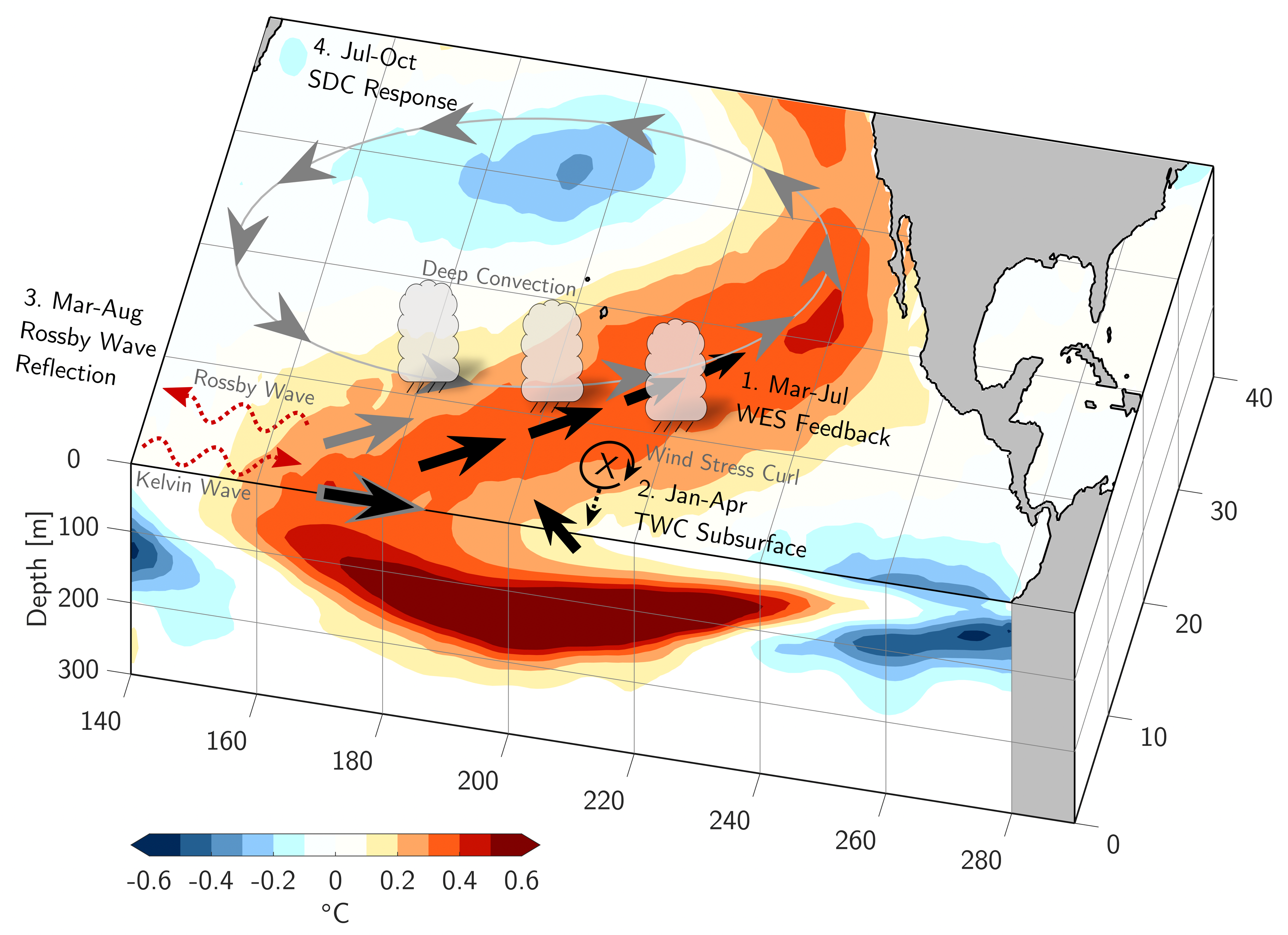
In each of the world’s tropical ocean basins, year-to-year climate variability is primarily governed by fluctuations in the zonal sea surface temperature (SST) gradient and zonal surface winds. These variations consist of recognizable climate modes, such as the El Niño-Southern Oscillation (ENSO) in the tropical Pacific and Atlantic Niño in the tropical Atlantic.
While meridional or interhemispheric fluctuations of tropical SST and surface winds—known as Meridional Modes—are less dominant, they still lead to significant impacts on humans and society by directly altering regional rainfall patterns over land and by modulating the timing and intensity of other climate modes like ENSO. The different flavors of Meridional Modes are discussed in the Expert Guidance section of this page.
Key Strengths
The meridional mode indices provide a concise way of characterizing interannual climate variability in the tropical ocean basins
Provide additional insight into tropical climate variability and its impact on society beyond ENSO
Key Limitations
The temporal homogeneity of the indices is only as good as that of the underlying input data
Filtering and pre-processing steps may not entirely remove the signals of ENSO and/or global trends
Expert User Guidance
The following was contributed by Dillon Amaya, December 2020:
In each of the world’s tropical ocean basins, year-to-year climate variability is primarily governed by fluctuations in the zonal sea surface temperature (SST) gradient and zonal surface winds. These variations consist of recognizable climate modes, such as the El Niño-Southern Oscillation (ENSO) in the tropical Pacific and Atlantic Niño in the tropical Atlantic.
While meridional or interhemispheric fluctuations of tropical SST and surface winds—known as Meridional Modes—are less dominant, they still lead to significant impacts on humans and society by directly altering regional rainfall patterns over land and by modulating the timing and intensity of other climate modes like ENSO. The different flavors of Meridional Modes are discussed below.
Atlantic Meridional Mode
The Atlantic Meridional Mode (AMM) represents seasonal-to-decadal fluctuations in the interhemispheric SST gradient within the tropical Atlantic (Figure 1). An AMM event begins its lifecycle in boreal winter, when stochastic weather noise from the Northern Hemisphere extratropics extends into the subtropics and influences the strength of the trade winds. This random atmospheric variability can be associated with passing extratropical storms or large-scale climate modes such as the North Atlantic Oscillation (NAO). Once these SST anomalies grow large enough, they then couple back to the atmosphere and drive cross-equatorial surface wind anomalies that bend in a characteristic C-shape pattern going from the Southern Hemisphere to the Northern Hemisphere as they feel the changing Coriolis force. During a positive AMM phase (i.e., warm tropical North Atlantic), the anomalous cross-equatorial winds strengthen the climatological Southern Hemisphere easterlies while simultaneously weakening the Northern Hemisphere easterlies, leading to increased evaporation and cooler SSTs in the Southern tropics and decreased evaporation and warmer SSTs in the Northern tropics. These Wind-Evaporation-SST (WES; Xie & Philander, 1994) interactions drive a positive feedback and reinforce the anomalous meridional SST gradient, which tends to peak in boreal spring.
In addition to maintaining an interhemispheric SST gradient, WES feedback effectively propagates SST and surface wind anomalies southwestward from the subtropical North Atlantic down into the deep tropics (Amaya et al., 2017). As a result, the AMM (and other Meridional Modes) represent an important pathway by which random atmospheric variability in the extratropics can teleconnect into the deep tropics and influence climate.
In particular, warm tropical North Atlantic SSTs associated with an AMM event can anchor the seasonally migrating Intertropical Convergence Zone (ITCZ) over the open ocean, significantly impacting regional rainfall over land and depleting water resources in Northeast Brazil and the African Sahel (Folland et al., 1986; Hastenrath & Heller, 1977). Additionally, the AMM has been shown to affect tropical Atlantic hurricane activity (Vimont & Kossin, 2007).
North and South Pacific Meridional Mode
While the AMM primarily evolves in the North tropical Atlantic, the Meridional Modes of the Pacific basin have Northern and Southern Hemisphere counterparts, known as the North Pacific Meridional Mode (NPMM; Chiang & Vimont, 2004) and the South Pacific Meridional Mode (SPMM; Zhang et al., 2014). The NPMM (Figure 2) and the SPMM (Figure 3) are very similar to the AMM in that they represent seasonal-to-decadal fluctuations in the local meridional SST gradient. As a result, they evolve in much the same way, emerging as a response to stochastic atmospheric forcing (either from the North Pacific Oscillation; NPO, or the South Pacific Oscillation; SPO) during the hemispheric winter. The NPMM and SPMM are also amplified by a positive WES feedback, which provides a similarly effective physical pathway for climate teleconnections from extratropics to the deep tropics (Amaya, 2019).
Many studies have linked the NPMM to the timing, intensity, and flavor (i.e., Central Pacific vs. Eastern Pacific) of ENSO events. In particular, research has shown that a positive or warm NPMM event peaking in boreal spring can lead to the development of an El Niño in the following winter, and vice versa for cold NPMM events. It is thought that NPMM events account for about 15-30% of observed boreal winter ENSO variability, with the 2015 NPMM playing a particularly important role in driving the extreme 2015/2016 El Niño (Amaya et al., 2019). The influence of the NPMM on ENSO stems from several oceanic and atmospheric pathways (Figure 4) that push the aggregated statistics of stochastic tropical noise (e.g., westerly wind events and/or equatorial subsurface ocean heat content) towards ENSO-favorable conditions. Improving our understanding of NPMM-ENSO interactions may improve long-lead ENSO prediction during a time of year in which forecasting models have historically struggled (e.g., the Spring Predictability Barrier).
The SPMM’s connection to ENSO is less clear. Some studies have shown that the SPMM interacts with ENSO events in much the same way as the NPMM, via a WES feedback-driven propagation of surface wind anomalies that leads to ENSO-favorable westerly wind events along the equator (You & Furtado, 2017, 2018; H. Zhang et al., 2014). However, recent research has also suggested that the SPMM need not “trigger” ENSO through equatorial ocean dynamics in order to significantly modulate its amplitude. Instead, the SPMM may act as a thermally driven source of eastern equatorial SST anomalies that constructively or destructively interferes with an existing ENSO event by modifying the effectiveness of latent heat flux damping (Larson et al., 2018; Okumura, 2013). Regardless, there is no clear consensus on the physical mechanisms that connect the SPMM to ENSO.
Finally, in addition to its impacts on ENSO, the NPMM has been linked to changes in tropical cyclone activity in the Western North Pacific , the Northeast Pacific, and the North Atlantic (Gao et al., 2018; Murakami et al., 2017; W. Zhang et al., 2018).
Meridional Mode Indices
Meridional Modes are typically defined by performing a Maximum Covariance Analysis (MCA) on monthly mean SST and the surface wind components (Amaya, 2019; Chiang & Vimont, 2004). Prior to calculating the MCA, both the SST and wind data are usually pre-processed to remove the effects of long-term trends (i.e., detrending), and to remove the influence of ENSO by linearly regressing out a measure of ENSO variability (typically the Cold Tongue Index or the first EOF of tropical Pacific SSTs). In the analysis presented here (Figures 1-3), we remove the first EOF of tropical Pacific (20˚S-20˚N, 140˚E-90˚W) SST. Additionally, it is common (but not required) to smooth the processed data with a 3-month running mean. The Meridional Modes are then defined as:
AMM: MCA1 of pre-processed SST/wind data in the domain 22˚S-32˚N, 74˚W-African coastline (Figure 1)
NPMM: MCA1 of pre-processed SST/wind data in the domain 20˚S-30˚N, 175˚E-95˚W (Figure 2)
SPMM: MCA1 of pre-processed SST/wind data in the domain 35˚S-20˚S, 180˚-70˚W* (Figure 3)
*There is no clear consensus on the SPMM domain, which varies slightly latitude and longitude. throughout the literature.
The MCA analysis results in two Expansion Coefficients (ECs), which represent the time variability of the SST and surface wind fields, respectively. The AMM, NPMM, and SPMM spatial patterns are then typically taken to be the regression of the pre-processed data on their respective normalized SST ECs, although the regressions could be made with the wind EC as well.
When wind data are not available, the Meridional Modes may be alternatively defined through an Empirical Orthogonal Function (EOF) analysis of similarly pre-processed SST data as follows:
AMM: EOF1 of pre-processed SST data in the domain 0˚-30˚N, 75˚W-African coastline
NPMM: EOF1 of pre-processed SST data in the domain 20˚S-30˚N, 175˚E-95˚W
SPMM: EOF1 of pre-processed SST/wind data in the domain 35˚S-20˚S, 180˚-70˚W*
*This definition is sensitive to domain choice. For example, if you choose 35˚S-10˚S, 180-70˚W instead, then the SST-only index is only moderately correlated with the SPMM’s traditional MCA SST EC.
These SST-only EOF-based indices of the Meridional Modes are highly-correlated with the traditional MCA-based SST ECs from 1979-2018 (red vs dark gray timeseries in panel b of Figures 1-3), with correlations of R = 0.97, 1, and 0.96 for the AMM, NPMM, and SPMM, respectively.
Pathways Forward
Meridional Modes are an important component of the climate system that significantly contribute to the overall variability of the tropical ocean-atmosphere state. Despite recent advances in this understanding, however, there are a number of outstanding questions that should be addressed. They include, among others:
- What is the role of tropical noise (e.g., the MJO, the build-up or discharge of subsurface ocean heat content, teleconnections from other basins) in modulating the efficacy of the Meridional Modes in impacting tropical climate?
- What role do Meridional Modes play in tropical decadal climate variability?
- How will Meridional Modes and the coupled processes that drive their evolution (e.g., WES feedback) change in the future?
- How important are Meridional Modes in communicating high-latitude climate perturbations (e.g., aerosols or Arctic sea ice loss) to the deep tropics?
Additionally, while the SST-only indices presented here strongly capture the variability of each Meridional Mode in the recent historical period, a more thorough analysis is needed with a longer observational record. It may be that these SST-only definitions provide a more reliable representation of the Meridional Modes, particularly in earlier decades when wind data is comparatively sparse, especially throughout the South Pacific and South Atlantic. We encourage future studies investigating the utility of SST-only Meridional Mode indices throughout the observational record.
##
Cite this page
Acknowledgement of any material taken from or knowledge gained from this page is appreciated:
Amaya, Dillon & National Center for Atmospheric Research Staff (Eds). Last modified "The Climate Data Guide: Meridional Modes and their indices.” Retrieved from https://climatedataguide.ucar.edu/climate-data/meridional-modes-and-their-indices on 2025-07-15.
Citation of datasets is separate and should be done according to the data providers' instructions. If known to us, data citation instructions are given in the Data Access section, above.
Acknowledgement of the Climate Data Guide project is also appreciated:
Schneider, D. P., C. Deser, J. Fasullo, and K. E. Trenberth, 2013: Climate Data Guide Spurs Discovery and Understanding. Eos Trans. AGU, 94, 121–122, https://doi.org/10.1002/2013eo130001
Key Figures
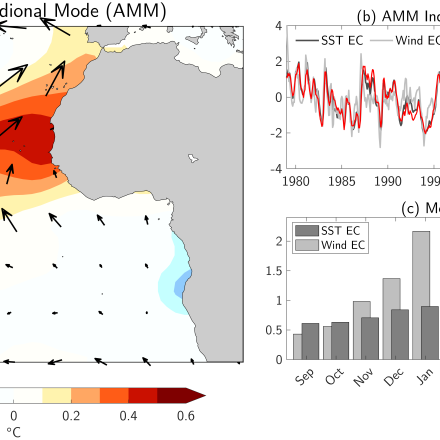
Figure 1 The Atlantic Meridional Mode (AMM). (a) MCA1 of pre-processed monthly mean SST and surface wind anomalies in the tropical Atlantic. Patterns depict regressions onto the normalized SST Expansion Coefficient (EC) timeseries. (b) Normalized AMM SST (dark gray) and wind (light gray) ECs. The red curve shows an alternative AMM index based on EOF analysis of pre-processed monthly mean SST anomalies. First number in title is the covariance explained for MCA1, second number is the correlation between the SST and wind ECs, and the third number is the correlation between the SST EC and SST-only EOF PC. (c) Monthly variance of the SST and wind ECs. The monthly variance for the SST-only EOF PC is very similar to the SST EC monthly variance (not shown). See text for details of the pre-processing. Monthly mean SST data is from ERSSTv5 and monthly mean 10m wind components are from ERA5, both from 1979-2018. All data was subject to the pre-processing steps described above. (contributed by D. Amaya)
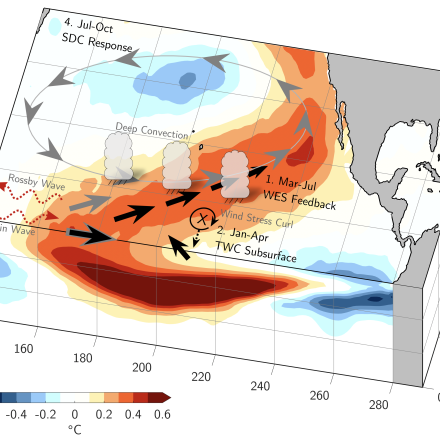
Figure 4 Schematic illustration of the primary physical pathways linking the NPMM to ENSO. They include: 1. A Wind-Evaporation-SST (WES) feedback driven propagation of surface anomalies onto the equator during boreal spring (solid black arrows), 2. Trade Wind Charging (TWC) of equatorial subsurface heat content by NPMM-related surface wind stress curl anomalies in boreal winter and early spring (black circular/dashed arrows and x/z shading), 3. The reflection of NPMM-forced ocean Rossby waves off the western boundary in boreal summer (red dashed arrows), and 4. A Gill-like atmospheric circulation pattern associated with an anomalous ITCZ shift in boreal summer and fall known as the Summer Deep Convection (SDC) response (clouds and solid gray arrows/lines). Each mechanism provides an opportunity for North Pacific ocean-atmosphere anomalies to influence ENSO development, either by altering off-equatorial ocean circulation or by generating zonal wind stress anomalies along the equator. Arrows not to scale.
Other Information
user choses the surface wind and SST datasets
depends on parent dataset(s)
- Amaya, D. J., 2019: The Pacific Meridional Mode and ENSO: a Review. Curr Clim Change Rep, 5, 296–307, https://doi.org/10.1007/s40641-019-00142-x.
- Amaya, D. J., M. J. DeFlorio, A. J. Miller, and S.-P. Xie, 2016: WES feedback and the Atlantic Meridional Mode: observations and CMIP5 comparisons. Clim Dyn, 49, 1665–1679, https://doi.org/10.1007/s00382-016-3411-1.
- Amaya, D. J., Y. Kosaka, W. Zhou, Y. Zhang, S.-P. Xie, and A. J. Miller, 2019: The North Pacific Pacemaker Effect on Historical ENSO and Its Mechanisms. Journal of Climate, 32, 7643–7661, https://doi.org/10.1175/jcli-d-19-0040.1.
- Chiang, J. C. H., and D. J. Vimont, 2004: Analogous Pacific and Atlantic Meridional Modes of Tropical Atmosphere–Ocean Variability*. Journal of Climate, 17, 4143–4158, https://doi.org/10.1175/jcli4953.1.
- Folland, C. K., T. N. Palmer, and D. E. Parker, 1986: Sahel rainfall and worldwide sea temperatures, 1901–85. Nature, 320, 602–607, https://doi.org/10.1038/320602a0.
- Gao, S., L. Zhu, W. Zhang, and Z. Chen, 2018: Strong Modulation of the Pacific Meridional Mode on the Occurrence of Intense Tropical Cyclones over the Western North Pacific. J. Climate, 31, 7739–7749, https://doi.org/10.1175/jcli-d-17-0833.1.
- Hastenrath, S., and L. Heller, 1977: Dynamics of climatic hazards in northeast Brazil. Q.J Royal Met. Soc., 103, 77–92, https://doi.org/10.1002/qj.49710343505.
- Larson, S. M., K. V. Pegion, and B. P. Kirtman, 2018: The South Pacific Meridional Mode as a Thermally Driven Source of ENSO Amplitude Modulation and Uncertainty. J. Climate, 31, 5127–5145, https://doi.org/10.1175/jcli-d-17-0722.1.
- Murakami, H., and Coauthors, 2017: Dominant Role of Subtropical Pacific Warming in Extreme Eastern Pacific Hurricane Seasons: 2015 and the Future. J. Climate, 30, 243–264, https://doi.org/10.1175/jcli-d-16-0424.1.
- Okumura, Y. M., 2013: Origins of Tropical Pacific Decadal Variability: Role of Stochastic Atmospheric Forcing from the South Pacific*. Journal of Climate, 26, 9791–9796, https://doi.org/10.1175/jcli-d-13-00448.1.
- Vimont, D. J., and J. P. Kossin, 2007: The Atlantic Meridional Mode and hurricane activity. Geophys. Res. Lett., 34, https://doi.org/10.1029/2007gl029683.
- XIE, S.-PING., and S. G. H. PHILANDER, 1994: A coupled ocean-atmosphere model of relevance to the ITCZ in the eastern Pacific. Tellus A, 46, 340–350, https://doi.org/10.1034/j.1600-0870.1994.t01-1-00001.x.
- You, Y., and J. C. Furtado, 2017: The role of South Pacific atmospheric variability in the development of different types of ENSO. Geophys. Res. Lett., 44, 7438–7446, https://doi.org/10.1002/2017gl073475.
- You, Y., and J. C. Furtado, 2018: The South Pacific Meridional Mode and Its Role in Tropical Pacific Climate Variability. J. Climate, 31, 10141–10163, https://doi.org/10.1175/jcli-d-17-0860.1.
- Zhang, H., A. Clement, and P. Di Nezio, 2014: The South Pacific Meridional Mode: A Mechanism for ENSO-like Variability. Journal of Climate, 27, 769–783, https://doi.org/10.1175/jcli-d-13-00082.1.
- Zhang, W., G. Villarini, G. A. Vecchi, and H. Murakami, 2017: Impacts of the Pacific Meridional Mode on Landfalling North Atlantic tropical cyclones. Clim Dyn, 50, 991–1006, https://doi.org/10.1007/s00382-017-3656-3.